CLASSE’s eBook provides an in-depth look at their critical role in globally-significant particle physics experiments.
The Cornell Laboratory for Accelerator-based ScienceS and Education (CLASSE) at Cornell University has participated in particle physics experiments over many years. The team was involved in developing the CLEO detector, they help build and operate the Compact Muon Solenoid (CMS) detector, and participated in the Fermilab Muon g-2 experiment. CLASSE’s data analysis efforts encompass Standard Model Higgs and top quark measurements, as well as searches for new physics such as supersymmetry.
The CLEO collaboration operated the CLEO high-energy physics detector from 1979 to 2008, recording the particles produced in electron-positron collisions at the Cornell Electron-positron Storage Ring (CESR). CLEO studied the production and decay of bottom and charm quarks, as well as tau leptons, to search for new phenomena beyond the Standard Model of particle physics.
The CMS experiment is a large general-purpose particle physics detector. Its goal is to record the results of extremely high-energy proton collisions, using this data to investigate a wide range of physics.
Muon g-2, which uses Fermilab’s powerful accelerators, will better understand the properties of the muon. This understanding will be used to probe the Standard Model of particle physics. The accelerators are used to explore the interactions of muons with a strong magnetic field in empty space.
This eBook, written by CLASSE’s Professor Anders Ryd, outlines the role of the team in these groundbreaking particle physics experiments and provides a detailed outlook on how the experiments have progressed over the years. The experimental particle physics group currently consists of six faculty members: J. Alexander, L. Gibbons, R. Patterson, A. Ryd, J. Thom-Levy, and P. Wittich. This work is supported by the U.S. National Science Foundation (NSF) and the U.S. Department of Energy (DOE).
Experimental particle physics at Cornell
CLASSE has a long history in experimental particle physics. For about 30 years, Cornell operated the CESR accelerator to study beauty, charm, and tau physics with the CLEO detector. Today, the experimental particle physicists at CLASSE participate in experiments based at CERN and Fermilab. We still develop and build experimental apparatus at the laboratory here at Cornell.
CLASSE is collaborating with groups from around the world, to help build and operate the Compact Muon Solenoid (CMS) detector, to analyse the data produced by it. The team, currently consisting of faculty members, graduate students, postdoctoral researchers, and undergraduate students, is responsible for operating and maintaining aspects of the pixel detector and its future upgrades, and for certain parts of the CMS software. Data analysis efforts by the group include studies of the Higgs boson, top quark measurements, and searches for long lived particles beyond the Standard Model.
CLEO
Following the discovery of the b-quark in 1977 at Fermilab the plan for an electron-positron collider at energies around the b-quark pair production threshold was focused and CESR, Cornell Electron-positron Storage Ring, was completed in 1979 with the first incarnation of the CLEO detector. Over the next 25 years, many upgrades and improvements to both CESR and CLEO were carried out. The instantaneous luminosity was increased from 1030 cm-2/s to 1033 cm-2/s – an improvement of three orders of magnitude. This included many firsts for particle accelerators.
The CLEO detector was upgraded in several steps to provide improved capabilities. The original CLEO detector explored different detector concepts and helped guide the upgrade of the CLEO II detector in the late 1980s. The detector concept of CLEO II with a low mass inner tracker, followed radially outward by an electromagnetic calorimeter, and a muon detector using a return yoke for the strong solenoid magnetic field, has been closely followed by several other experiments, e.g., BABAR and Belle. The CMS detector, discussed below, is similar in concept, though significantly larger for the higher energies at the LHC. The CLEO II detector is shown in Fig. 1.
In 2003, CESR and CLEO were reconfigured to operate at the charm threshold to study the properties of charmed mesons. CESR was equipped with wiggler magnets to increase the damping at low energy to increase the luminosity near the charm threshold. The CLEO-c detector was instrumented with a low-mass drift chamber closest to the interaction point.
Following the discovery of the Y(4S) in 1980, the physics programme with the CLEO experiment focused on flavor physics with bottom and charm quarks as well as tau physics. There were several significant discoveries and impressive progress was made. A few important milestones are discussed here.
The first exclusive reconstruction of B-mesons in 1983. The 1990 observation of semileptonic b→uµν decays which showed that Vub was non-zero. This opened the possibility for CP violation in the Standard Model of particle physics and motivated the programme of studies at the BABAR and Belle B-factories.
The first evidence in 1993 for the decay B→K*,2 a candidate event display is shown in Fig. 2. The B→K*, decay is interesting as it proceeds via a loop diagram, known as a Penguin diagram. This transition does not occur at the tree level in the Standard Model. The loop consists of a W boson and a top quark. When this decay was observed in 1993, the top quark had not yet been observed and the decay B→K*, provided indirect evidence for the top quark.
The charm programme with the CLEO-c detector studied the properties of D and Ds mesons in detail. This included measurements of CKM matrix elements Vcd and Vcs. The measurements of the Ds→µν decay allowed the direct measurement of fDs which provided an important calibration point for Lattice QCD calculations.
The CMS experiment
Scientists at CLASSE joined the CMS experiment at CERN in 2005 as the operation of CESR for particle physics was nearing an end. Our physics goals shifted from flavor physics in bottom and charm physics to searches for new physics at the highest energies available. We joined the CMS experiment about five years before data taking started. We contributed to the development of the software framework, CMSSW, used for data processing, the online software for the operation of the pixel detector, and the operation of the electromagnetic calorimeter and trigger system.
One of the areas we focused on during the first LHC Run, from 2010 to 2012, was the search for super symmetry. The much higher energy of the LHC compared to the earlier Tevatron provided a huge increase in the reach for super symmetry. We participated in the searches for third generation SUSY with top and bottom quarks in the final states.3 We set limits on gluino masses that were significantly more restrictive than earlier searches, see Fig. 3.
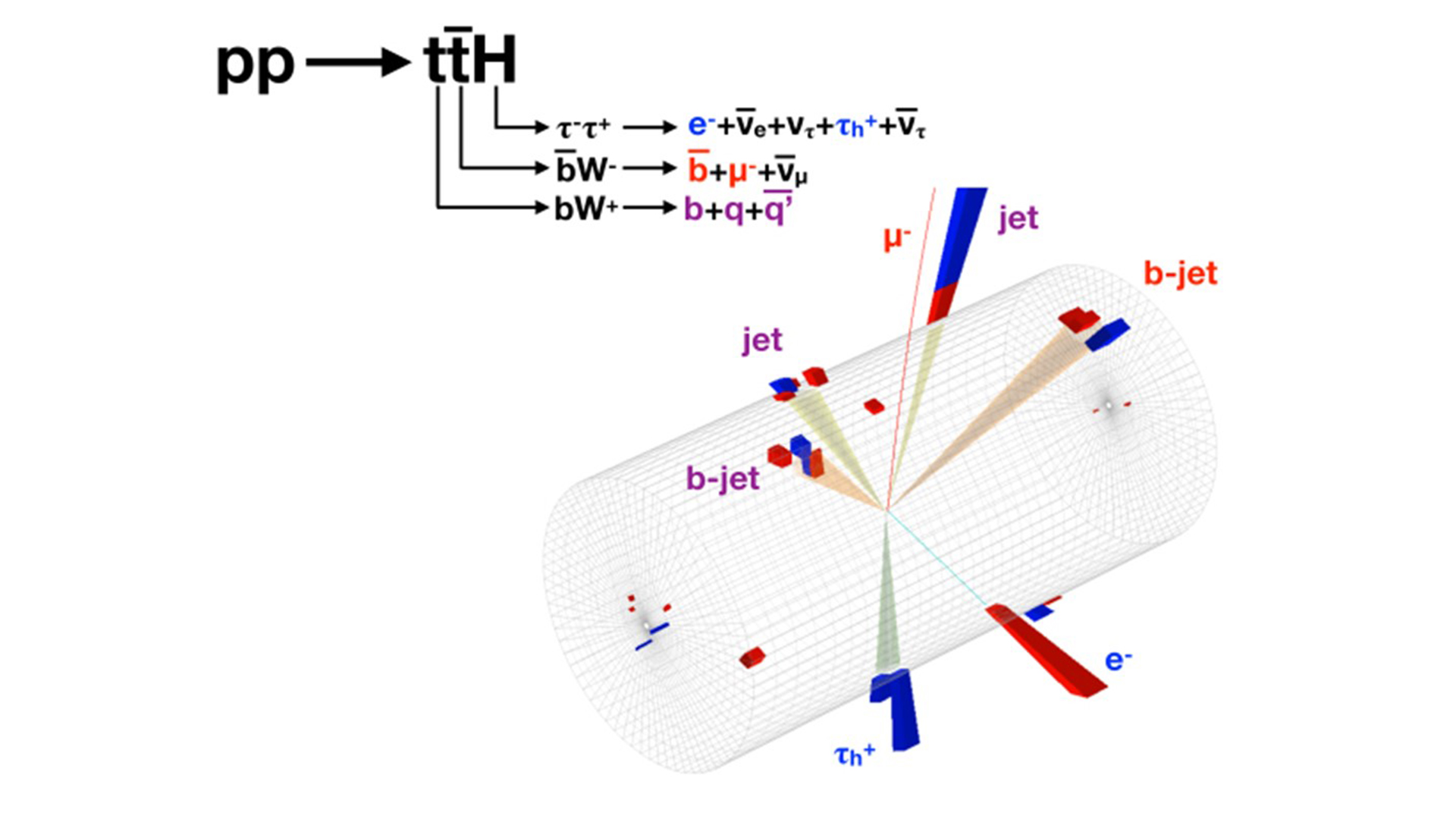
We also participated in searches for long lived particles. One analysis searched for long lived particles that decayed in the detector and give rise to jets that originate away from the interaction point.
Since soon after joining CMS in 2005, the group at Cornell has been involved with the plans for a major upgrade of CMS. The LHC will be upgraded to significantly increase the luminosity for the High-Luminosity LHC (HL-LHC). The HL-LHC plans to deliver an integrated luminosity to the experiments that are about an order of magnitude larger than what will be delivered before the upgrades. The HL-LHC upgrades will allow for detailed studies of the Higgs boson. One key goal is the measurement of the Higgs self-coupling. This will require the highest possible luminosity from the LHC in combination with detectors that can extract this rare signal. For the HL-LHC operation, the CMS tracking detectors will need to be replaced as they will have reached their end-of-life due to radiation exposure. The new detectors to be installed for the HL-LHC operation will need to be significantly more radiation hard to survive, have higher granularity to be able to separate the particles in the dense hit environment, and have increased readout bandwidth to handle the large data volume. In addition, we want to extend the forward tracking capabilities out to ŋ=4.0, corresponding to an angle of about 2.0 degrees from the beamline, and add the capability of using the data from the tracker in the hardware trigger system.
The Cornell CMS group and staff from CLASSE are major leaders of the NSF-funded upgrades for the HL-LHC. Cornell manages the MREFC (Major Research Equipment and Facility Construction) award from the NSF which provides the US support for the upgrades of the Pixel Detector, readout electronic upgrades for the Muon and Barrel Calorimeter detectors, and the Muon and Track Trigger. For the Pixel Detector, Cornell is mainly responsible for the mechanics and integration, see Fig. 5, with contributions to sensors and electronics. For the Track Trigger, Cornell, jointly with Boston University, is responsible for the hardware platform, Apollo, as well as a main contributor to the track-finding algorithm and the firmware and software. The ability to find tracks in the first hardware level of the trigger is a unique new capability for the upgraded CMS detector that will provide a new handle to manage the trigger rates at HL-LHC.
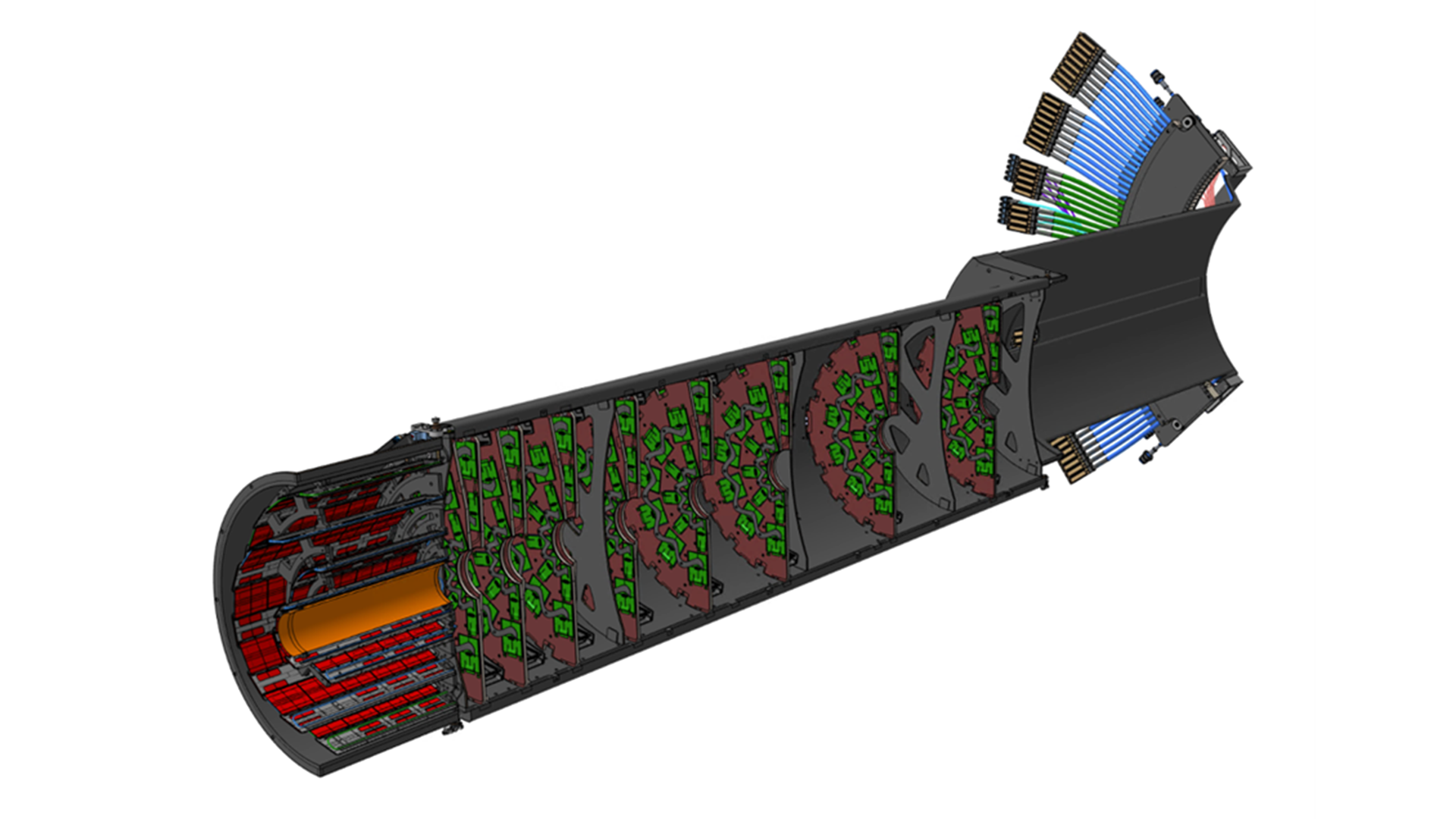
Muon g-2
In addition to searching directly for the production of new types of particles and their associated interactions, one can look for evidence of such particles through precise measurement of quantities that can be accurately predicted within the Standard Model framework. The muon magnetic moment, and in particular the deviation of its ‘g-factor’ from the relativistic quantum mechanics value of 2, provides such a probe. A measurement of g-2 at BNL from circa 2000 had persisted in showing a roughly 3.5 standard deviation discrepancy with the Standard Model prediction. The Fermilab Muon g-2 experiment set out to remeasure g-2 with a factor of four improvement in precision.
Cornell joined this effort and has made key contributions in electronics and muon beam delivery systems for the experiment, and in the reconstruction and analysis of the data to determine g-2. The first result from the Fermilab experiment, released in 2021, confirmed the BNL result.4 Since that time, new experimental measurements that provide input to the Standard Model prediction for g-2, as well as new techniques for calculating g-2 (lattice QCD), have placed the Standard Model prediction in a state of flux. Cornell is continuing to participate in the analysis of the muon g-2 data from Fermilab.
References
- “The CLEO II detector”, Y. Kubota et al. (CLEO Collaboration), Nucl. Instr. and Meth. A320, 66 (1992)
- “Evidence for penguin-diagram decays: First observation of B→K*(892)γ”, R. Ammar et al. (CLEO Collaboration), Phys. Rev. Lett. 71, 674 (1993)
- “Search for supersymmetry in events with b-quark jets and missing transverse energy in pp collisions at 7 TeV”, S. Chatrchyan et al. (CMS Collaboration), PRD 86, 072010 (2012)
- “Measurement of the Positive Muon Anomalous Magnetic Moment to 0.46 ppm”, B. Abi et al. (Muon g−2 Collaboration), Phys. Rev. Lett. 126, 141801 (2021)