Physicists at Jefferson Lab use resonating protons to gain insight into the Universe that existed just after the Big Bang.
The atomic nuclei of all elements in the Universe consist of protons and neutrons. From the pioneering measurements of Robert Hofstadter and his co-workers in the 1950s, it became evident that protons are not point-like particles. Protons have a finite size, on the order of one femtometre, which makes the size of the proton to be around 100,000 times smaller than that of the atom.
Electrons, on the other hand, are point-like particles that have the opposite charge of the proton. By directing high-energy beams of electrons onto protons by using a particle accelerator and by examining the directions in which the electrons were deflected, we can measure the inside of protons.
Taking pictures of the inside of protons
We can draw an analogy to spectroscopy from optics. Just as light reveals the structure of atoms, we can similarly infer the spatial structure of the objects under investigation from the diffraction pattern of the reflected electrons. By analogy, if we direct a laser through a pinhole, a pattern will be formed with a central bright spot surrounded by a series of concentric rings. Going outwards from the centre, the rings will become fainter. Examining this diffraction pattern provides telltale information on the size and shape of the pinhole. Electrons obey quantum mechanics and possess a wavelike nature. As their energy increases, the wavelength gets smaller. So, high-energy electrons of a billion electron volts (GeV), requiring powerful particle accelerators, will have wavelengths comparable to the size of the proton and will thereby provide spatial information on the inside of protons.
In fact, a proton’s structure is quite complex, compelling scientists to abandon the notion that protons are elementary particles. Starting with Hofstadter’s Nobel-prize-winning research, electron scattering experiments revealed that protons have a rich inner structure formed around three components, later named quarks, and possess distinct distributions. The discovery of unexpectedly large numbers of so-called deep inelastic scattering events gave direct proof that the quarks really exist inside of the proton. These quarks are bound so strongly that no quark can ever leave a proton. In the intervening years, many electron scattering measurements have been performed to provide one-dimensional pictures of the distribution of the quarks.
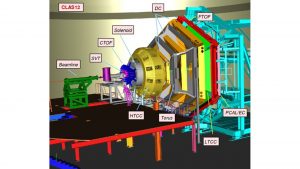
Resonating protons
Since the middle of the last century, physicists have employed accelerators to create beams impinging onto proton targets. They have found that protons may resonate just like striking a bell at its sweet spot. If the bell vibrates just right, there will be an ensuing tone. A proton is clearly not a bell, but it will vibrate when a particle strikes it at just the right energy or frequency. These characteristic tones of the proton are called resonances, and the quark model can explain their existence. A proton is a composite system of three quarks bound together by the strongest force known in nature, the strong force. The ground state is the lowest-energy state of the proton. When a proton is excited by a particle beam into a higher-energy state, its quarks rotate and vibrate against each other, exhibiting resonance characteristics. In 1952, Enrico Fermi saw the first resonance, the ∆, which is one-third times as massive as the proton and can be described as a magnetically excited state. Another very prominent resonating proton, called the Roper, is now understood as a radial excitation of the proton ground state. This is like the breathing mode frequency, wherein there is a variation in the distance of the quarks from each other, going in and out.
Advances in theory, electron accelerator, and detector technology over the past few decades have provided a novel way to describe proton structure in three dimensions, two in space and one in momentum, from electron scattering. This has further opened a new avenue of research and has led to three-dimensional pictures of the ground-state proton. Still, little is known about the three-dimensional structure of resonating protons. 3D imaging, also called nucleon photography, can potentially delineate the internal structure of protons completely. This is not unlike tomography in 3D imaging. These 3D images are captured by the distribution functions that encode the internal properties. Ultimately, the goal is to unambiguously extract and fully understand the distributions of quarks inside the proton. This requires high-precision measurements, good data sets, and powerful analytical tools.
New experiments and technologies at Jefferson Lab in the US
Currently, experiments are being conducted at the U.S. Department of Energy’s Thomas Jefferson National Accelerator Facility (Jefferson Lab). From the world-class Continuous Electron Beam Accelerator Facility (CEBAF), high-energy electron beams are focused onto resonating protons surrounded by large detectors such as CLAS12 in Jefferson Lab’s Experimental Hall B. CLAS stands for CEBAF large acceptance spectrometer, and the 12 represents the recent upgrade to Jefferson Lab from 6 GeV to 12 GeV beam energy. CEBAF is a DOE Office of Science user facility that supports the research of more than 1,800 nuclear physicists worldwide. The CLAS12 detector is a large-acceptance magnetic spectrometer based upon a superconducting torus magnet that provides a largely azimuthal field and a solenoid magnet with full azimuthal coverage. The complex instrument can operate at high luminosities. The higher the luminosity, the more likely an electron will strike the inside of protons. Since the proton is 100,000 times smaller than the atom, it takes many impinging electrons to contact the minute proton.
The capabilities of CLAS12 are being used in a broad programme to study the structure and interactions of fundamental particles. Resonating protons live for around a trillionth of a trillionth of a second, or about the time it takes for light to cross the distance of a proton. These resonances will typically relax into a ground state by emitting a quark-antiquark state — termed a meson. CLAS12 can track newly formed particles such as these mesons and the scattered electrons moving away from the target, allowing the reconstruction of resonating protons. It can also record the particle’s flight times with a precision better than a tenth of a billionth of a second. Therefore, like an optical instrument but on a much larger scale, the spectrometer provides a view into the invisible realm inside of the proton. The CLAS12 detector’s output is recorded 20,000 times per second. It is stored in hundreds and thousands of terabytes of data files for each experiment performed by the CLAS Collaboration, which involves about 200 physicists from almost 50 institutions worldwide.
It typically takes years to analyse the data and to extract observables that are sensitive to the proton structure. To access the deeply hidden world of distribution functions, the electrons from CEBAF impact the proton inside the hydrogen ‘target’ in the centre of the CLAS12 detector to excite the quarks further within and produce the proton resonances. These excitations are unbelievably short-lived, disappearing after ten to the power of − 24 seconds, one-millionth of one-billionth of one-billionth of a second. Still, they leave behind evidence of their fleeting existence. Multiple layers of advanced particle detector technologies are needed to identify the mesons produced in the collisions by measuring their properties, such as velocity, mass, and charge.
Modern artificial intelligence (AI) and machine learning methods are employed to support physicists, advance the development of software systems, improve experiments, and speed up the simulation and analysis processes. The CLAS Collaboration is at the forefront of scientific developments in its use of AI methods and tools, profoundly accelerating scientific knowledge acquisition, creating synergies across scientific areas, and improving international competitiveness. Modern physics research has already been transformed by AI techniques, and interfacing physics experiments with AI is expected to play an increasing role.
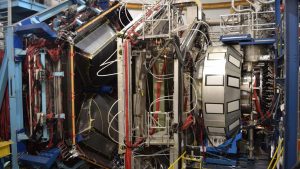
The strongest of all forces
The dynamics of the strong interaction, the fundamental force that holds the quarks inside the proton together, are very complicated, and so is the theory used to describe it: quantum chromodynamics (QCD). A great advantage of using electrons as a probe is that the theory of quantum electrodynamics, QED, precisely describes the electron interaction. QED is an incisive and precise tool, the crown jewel of theory. With such a precise tool, we can access the QCD properties of the proton resonances from the data of the experiments with CLAS12. One remarkable feature of QCD is that the force between quarks is small when they are close together but grows larger as they move apart. This is very different from what is known about the other basic forces in nature ─ gravitational, electromagnetic, and weak. Comparing measurements to QCD’s predictions is needed to test the theory, as the protons cannot be described from first principles. The calculations do not readily converge, and we cannot use valid approximations for weak fields.
Recent achievements stimulate a deep question about QCD: How does QCD generate the spectrum and structure of proton resonances? Relating the underlying theory of QCD to observations made at electron accelerators requires theoretical models with many different approaches. With a better understanding of how the quarks form resonances, these models can be tested, and critical insights into the basic building blocks of matter are gained.
The hot universe that existed just after the Big Bang
The laws of QCD and the increasing knowledge from the accelerator-based experiments can be applied to the early Universe. Space has been expanding since its creation, called the Big Bang, billions of years ago. As it expands, the Universe becomes less dense and cool. But when it was very young, it was a hot soup of fundamental particles called a quark-gluon plasma. This is a state where the quarks are not bound into protons but a plasma of freely moving quarks within a soup of gluons, where the gluons are the force particles that bind quarks together. The transition from the plasma to confined quarks began just microseconds after the Big Bang. Dramatic events occurred during that era in which strong QCD was born, and proton excitations were abundant. Within less than a millisecond, ground-state protons and their counterpart neutrons formed and eventually yielded the matter that we all are made of. While this stage in the evolution of the Universe cannot be recreated in any laboratory, one can make use of the QCD theory to describe what happened in the early universe and perform experiments at Jefferson Lab and other accelerators around the world to explore the processes in relative isolation. Because the proton resonances were the first states that the quarks formed, they contain important information for the understanding of the early Universe. The excitation structure of the proton is key for describing the dynamics of the universe: Resonating protons still ring like a bell as they did billions of years ago.
Since the experiments of Hofstadter and his colleagues in the 1950s, electron scattering has remained the most precise method for studying the proton, the dominant component of all visible matter in the Universe. In his Nobel lecture, he praised such research: “Over a period of time lasting at least two thousand years, Man has puzzled over and sought an understanding of the composition of matter. It is no wonder that his interest has been aroused in this deep question because all objects he experiences, including even his own body, are in a most basic sense special configurations of matter.”
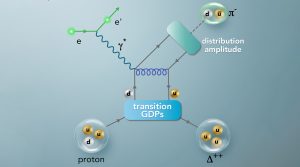
The recently studied processes of the three-dimensional structure of resonating protons were the first of its type and, therefore, opened a new field of research. More state-of-the-art experiments are planned at the Jefferson Lab to assess different characteristics of the processes. Each experiment like this adds more puzzle pieces to the picture of the hot era that existed just after the Big Bang and allows physicists to learn more about the history of the early Universe. Not all answers in science are known yet. With research and experiments underway, one can learn of the mystery of the strong force, what most of the Universe is made of, and what the ultimate fate of it will be.
Moreover, with the advent of the James Webb Telescope and its data on the nature of the Big Bang, galaxy formation, and the visible Universe, now more than ever, we need to understand what makes a proton a proton. Everything fits together, but it takes many approaches to reveal the nature of the quantumly small to the cosmologically large. The study of proton resonances links how protons are excited and how they evolved into existence and coalesced after the first moments of the beginning of the Universe.
Please note, this article will also appear in the sixteenth edition of our quarterly publication.