The Executive Director of SNOLAB, Dr Nigel Smith, spoke to The Innovation Platform about the facility’s science programme, most notably its neutrino activities.
A world-class science facility located deep underground, SNOLAB (the deepest clean room facility in the world) allows extremely rare interactions and weak processes to be studied. The science programme at SNOLAB is currently focused on sub-atomic physics, largely neutrino and dark matter physics. It seeks to enable, spearhead, catalyse and promote underground science, whilst inspiring both the public and future professionals in the field.
The Innovation Platform spoke to SNOLAB’s Executive Director, Dr Nigel Smith, about the facility’s science programme, most notably its neutrino activities, and some of the challenges involved – from shielding from cosmic radiation to developing detectors.
Could you begin by providing an outline of the science that takes place at SNOLAB? What makes it unique or different to other labs? How does it build on, for instance, the Sudbury Neutrino Observatory (SNO) solar neutrino experiment – in terms of both the infrastructure and the science?
SNOLAB is a deep underground research facility. We are hosted by the Vale mining company 2km down in their nickel mine in Sudbury, Ontario, Canada. The science that we do is generally related to astroparticle physics, which connects particle physics, cosmology and astronomy and is generally related to understanding subatomic particles, where they come from and their influence on the Universe. We need to be deep underground because we are running very sensitive experiments as we look for the ways in which these subatomic particles interact with our detectors. If these experiments were to be operated above ground, the very small, elusive, extremely rare signatures we are looking for would simply be swamped by cosmic radiation.
To overcome this, then, the infrastructure needs to be deep underground – which is a challenge in itself, not least because these are very large experiments (SNO, SNOLAB’s predecessor, was the scale of a 10 storey building, for instance). And yet, while being underground does help to shield from cosmic radiation, the material the detector is built from, as well as the material around it – for us, this is the rock of the mine – also has levels of radioactivity that would interfere with our observations. And while this normally isn’t an issue as they are such low levels of radioactivity, it can have a significant impact on the types of experiments we are undertaking.
We also have to make sure that our detectors are very clean – for instance, the radiation budget for the SNO experiment showed that if there were just two grams of dust in the detector (keeping in mind that this could be placed on the face of a coin), then we would not see the signals we were looking for.
SNOLAB, and labs like it, are located underground so as to produce a radioactively quiet environment which enables the search for rare or very small signatures that stem from the interactions of subatomic particles. And at SNOLAB, our experiments focus on studies of the neutrino, an elusive subatomic particle, and dark matter.
We know neutrinos exists because we have seen them; they are produced in prodigious numbers in the Sun, for instance. And we believe that dark matter exists because there appears to be more mass in the Universe than we can identify, and one solution to this would be that dark matter is a new type of sub-atomic particle, and this is something we are searching for.
The science programme we are pursuing at SNOLAB builds on the SNO detector, which used a kilotonne of heavy water and 10,000 light sensors designed to look for the interaction of neutrinos from the Sun in that detector. SNO was instrumental in solving what was known as the ‘solar neutrino problem’, which had existed for several decades. This stemmed from the Homestake experiment, located in a mine in South Dakota, which used a tank of cleaning fluid to look at the conversion of chlorine atoms to argon as a result of neutrino interactions. The results of this experiment suggested that there were fewer neutrinos coming from the Sun than the fusion models, which had been developed to explain how the Sun was burning, had predicted.
After some time, it transpired that the neutrinos themselves were the issue. That is, the fusion processes in the Sun creates one of the three ‘flavours’ of neutrino: the electron neutrino. But by the time they reached the Earth, they had morphed into one of the other flavours, the muon or tau, in a process now known as neutrino oscillation. SNO was instrumental in demonstrating that when the neutrinos of all different flavours were added together, they totalled the amount that were expected. This also demonstrated that the fusion models did indeed work, which, in addition to meaning that we correctly understand how the Sun burns, was important for fusion research on Earth. This work resulted in Arthur B. McDonald being co-awarded the 2015 Nobel Prize in Physics.
From the SNOLAB perspective, the work that was done by SNO to address the solar neutrino problem also demonstrated very clearly that it is possible to build and run such large, clean, complex detectors in an environment like a nickel mine. SNOLAB was thus proposed on the back of the SNO project’s success, and we now have a much broader science programme.
It has also been fascinating to observe how the infrastructure we have at SNOLAB also allows other, more diverse, research threads to develop. For instance, we have projects on genomics – one of which is looking at the impact of background radiation on cellular development, which is important for understanding low level radiation impact and damage, and another is looking at the metabolism of fruit flies as a model organism to develop a better understanding of the impact of such an environment on those who work below ground. Furthermore, there is a whole community looking at the deep subsurface biosphere and how life develops without being exposed to the Sun.
These are fascinating areas where the techniques we have developed in astroparticle physics can have an impact elsewhere, and we are now a truly multidisciplinary and international research facility that grew out of just one project.
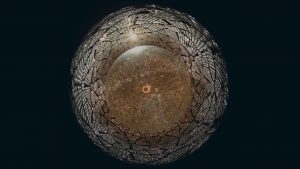
Can you tell me more about SNO+ and its efforts to make precise measurements of the solar neutrino spectrum? Why is this important? What challenges will you need to overcome to achieve this?
SNO+ is the follow up to the SNO detector which, as previously mentioned, used 1,000 tonnes of heavy water as a target for neutrinos coming from the Sun. By looking at neutrino interactions here, we were able to see how many neutrinos of each different flavour came from the Sun, and that measurement allowed us to solve the solar neutrino problem.
SNO+, however, has several science threads. And while they are all related to neutrinos, solar neutrinos are only one of them. In fact, the primary science goal of SNO+ is the search for neutrinoless double beta decay. Beta decay is a nuclear process whereby, in the nuclei of certain elements, a neutron decays into a proton, resulting in an electron and a neutrino being emitted. In double beta decay, this happens twice, thereby resulting in two electrons and two neutrinos being emitted. Neutrinoless double beta decay can occur if the neutrino is its own antiparticle, as this would mean the neutrinos can annihilate and all the energy would go into the two electrons. Thus, by measuring those two electrons it is possible to gain an understanding of the neutrinos themselves and, what is more, a better understanding of their mass. And, more importantly, it should be possible to tell whether the neutrino is a Majorana particle, that is, its own anti-particle.
To achieve this, we replaced the heavy water from the SNO experiment with normal ultra-pure water. We are now replacing the water with something called ‘liquid scintillator’, a material which gives off light, meaning that it is possible to see the scintillation as particles interact. This filling process has stalled as a result of the COVID-19 pandemic and restricted access to the lab and the experiments.
The scintillator is an oil called linear alkyl benzene, and SNO+ will use about 800 tonnes of this scintillating material. The energy threshold is lower for linear alkyl benzene when compared to the heavy water, and so we will be able to see lower energy interactions in the media. This will allow us to look at different parts of the solar neutrinos’ energy spectrum, which is important as there are certain nuclear fusion processes in the Sun which produce lower energy neutrinos as different fusion processes occur. By replacing the heavy water with liquid scintillator, we anticipate that we will see more of the various types of the Sun’s stellar fusion processes. SNO+ will look at the lower energy interactions and also potentially help to answer some of the questions that remain about stellar fusion, such as those concerning neutrino oscillation and how these processes take place in the presence of matter.
As a part of the planning for SNO+, there was a huge research campaign to discover how to dissolve a heavy metal into an organic fluid. That research has been concluded and we intend to dissolve tellurium into the fluid. This was chosen because one of the isotopes of this is tellurium-130, which undergoes the double beta decay process, meaning that we can look at the double electron signatures that occur from the neutrinoless double beta decay process.
Meanwhile, we will also explore neutrinos that come from the Earth, so-called ‘geoneutrinos’. SNOLAB is located in the centre of the North American continent, and it therefore ‘sees’ a different distribution of geoneutrinos than what is observed elsewhere, making the combined data more useful. We hope that this data will go on to result in a better understanding of the Earth’s crust and, potentially, the mantle.
SNO+ will also look at supernova neutrinos. Supernovae are relatively rare phenomena – it is thought that only two or three such events occur in our galaxy each century. But when they do happen, neutrinos are expelled from the core of the star – just as they are from the core of our own Sun. Thus, when and if we are able to detect a neutrino pulse coming from a supernova, they can teach us a lot about what is happening at the centre of the star before it explodes.
The light pulse from a supernova comes after the neutrino pulse, as the light is scattered in the plasma of the explosion, and the pulse is slowed down. This means that there is a time gap between the neutrino pulse and the optical pulse, and so by looking at the different flavours of neutrinos, we can obtain information about the processes that are occurring in the supernova and, what is more, we can give astronomers a warning that a supernova is under way.
Indeed, SNO+ will contribute to a global array called ‘SNEWS’ – the Supernova Early Warning System – which is a collection of global neutrino experiments that are waiting for a supernova to happen. When and if it does, and they see the signature, they will work together to confirm that it is indeed a supernova before providing an early warning to, for instance, optical astronomers. This is possible because we will be able to detect the neutrino pulse first, with potentially several hours before the astronomy community will be able to detect the light.
In addition to SNO+, we have another detector at SNOLAB called HALO. This recycles some of the neutron detectors and other materials from other older experiments, and its purpose is to also look at the neutrino signature from a supernova. It is quite a small, compact experiment that uses about 80 tonnes of lead, and there are plans to try to increase that to around 1,000 tonnes.
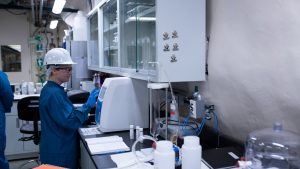
Other labs and experiments around the world are searching for neutrinoless double beta decay? How do you try to ensure that you are doing something different?
The process of neutrinoless double beta decay will only happen in a few isotopes. And one of the critical things will be to make sure that it has been observed in more than one of them. One reason for this is simply to verify the experimental results. SNO+ uses tellurium, but there are other detectors around the world that are using germanium-76 and xenon-136. By looking at double beta decay in these other isotopes, it will be possible to verify that this process is actually happening.
In addition to the systematic, experimental aspects, it is also important to note that there are elements of nuclear physics coming into the signal that we expect to see. In such an experiment, it is the half-life of the double beta decay process that is being measured. There is then a connection between the half-life being measured and the effective neutrino mass that is pulled out from measuring the double beta decay process. That translation contains elements of both nuclear and particle physics. Nuclear physics is also important here because we don’t fully understand the nuclear theory that would be applied, with certain models being used to translate this half-life to a measurement of the effective mass. And while the scatter is getting better, there are factors of ten between the various nuclear models that you can use to make this translation.
Because of this, it is important to have a whole set of nuclei so that the nuclear physics can be differentiated and so that the information you are interested can be extracted. That is the reason that more than one detector is important. And while there are a few experiments around the world similar to what we are doing at SNOLAB, it should also be noted that such projects are getting larger and more expensive, with some of the detectors currently being planned predicted to cost somewhere in the region of USD $200-250m, typically because the isotopes can be very expensive. This increase in scale and cost has resulted in an enhanced level of co-operation and co-ordination, not just between the labs but also between the international community and the funding agencies, to be able to support both the current programme and the next generation.
At SNOLAB, we have one cavity remaining to be filled and this is to be allocated to a second generation programme for neutrinoless double beta decay. Several projects have expressed an interest in putting a detector into SNOLAB, and we are working with funding agencies around the world as well as with the scientific community to decide which project will get selected and hopefully then come to SNOLAB. The scientific community is aware of the need for several of these systems to be up and running, and so the question really concerns the best location for them (which depends on the characteristics of the experiment in question).
We are also taking part in a dialogue with the US Department of Energy and its nuclear physics team who are building a process to select which projects the US is really interested in moving forward.
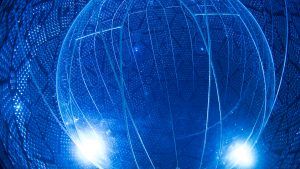
Looking to the future, what elements of SNOLAB’s science are you particularly excited about?
The two areas that we have already discussed, dark matter and neutrinoless double beta decay, are perhaps the most exciting. Everyone who works in this field wants to understand these two areas of astroparticle physics. Neutrinoless double beta decay would be a tremendous observation; it would not only give us information about neutrino mass but also its nature.
Research to observe neutrinoless double beta decay is thus pushing the boundary of the Standard Model. We know neutrinos have mass, and we know they must very light; but we don’t know what the individual neutrino masses are for any of the three flavours, and we don’t know the hierarchy of them, although we assume the electron is lightest and then muon and then tau, but we don’t know for sure. And so, by observing neutrinoless double beta decay, we hope to answer these questions and, more importantly, we hope to understand whether the neutrino is its own antiparticle; demonstrating that the neutrino is a Majorana fermion would provide a major piece of information to the field of particle physics.
Understanding dark matter is also a massive issue in contemporary astroparticle physics, cosmology, and particle physics. We know that there is more matter in the Universe than we expect due to the gravitational influences that we can see, and we know that this has an effect on the way the Universe has evolved and, indeed, on the way galaxies have formed. For instance, without dark matter, most galaxies would still not have formed at all. It is therefore vital to understand what this matter is and how it has influenced the evolution of the Universe.
The first direct observation of dark matter in our galaxy would be incredibly important for a whole slew of science because it connects the very big – how the Universe is evolving – to the very small (particle physics), and would see an entirely new area of particle physics begin to open up.
Of course, at SNOLAB we are also trying to support a much broader interdisciplinary programme. We have touched on the genomics research that is taking place here, for instance, and we are also looking to have societal impact. We are achieving this by joining a network of labs and universities which have come to explore how we can help society in light of the COVID-19 pandemic. And this is not only in terms of understanding the virus; we have been engaged in a project that is building a new type of ventilator, for instance. This is building on an old concept that dates back to the 1950s, but we are applying modern techniques and the things we have learned from building detectors and adapting them to the current crisis.
How important is outreach?
We are often asked why government supports fundamental research, and for this I answer with three components: knowledge, technology, and people. We have discussed the knowledge that is generated from the exciting physics and the way we want to generate a better understanding of the Universe. We have also discussed some of the technologies – from detectors and clean rooms to spinouts such as ventilators. But inspiring people is just as important and is one that national labs and universities need to continue to focus on.
National laboratories offer a relatively stable environment where there is space for expertise to develop to address some of the largest problems in contemporary science, and because of this, we can be a great source of inspiration. People are inspired by the work that we do, and this inspiration is crucial for the next generation of researchers, scientists, and engineers who can look at the big problems we are addressing, find them as fascinating as we do, and make the decision to forge their own careers in these areas. And that, of course, has wider societal benefits too.
Dr Nigel Smith
Executive Director
SNOLAB
+1 (705) 692 7000
info@snolab.ca
Tweet @SNOLABscience
www.snolab.ca
Please note, this article will also appear in the third edition of our new quarterly publication.