Professor Arthur Champagne, from the University of North Carolina – Chapel Hill and the Triangle Universities Nuclear Laboratory, describes a new accelerator facility for nuclear astrophysics.
Nuclear reactions and decays generate the energy that makes the stars shine. At the same time, these processes produce the elements that ultimately become the building blocks for new stars, planets, and life itself. For most of their lives, stars exist in an equilibrium between the outward pressure caused by nuclear burning and the inward pressure resulting from the gravitational attraction. Thus, changes in the thermodynamic conditions in the interior of a star can lead to dramatic changes in the stellar structure. These can be driven both by the rate of energy generation and also by variations in the chemical composition. In other words, nucleosynthesis drives stellar evolution.
The goal of nuclear astrophysics is a detailed understanding of the nuclear processes in stars. This information is an essential input to stellar models that in turn can be validated through observations. Such observations provide motivation for further nuclear studies and predictions of nucleosynthesis can in turn stimulate further observational studies. Almost all questions in astrophysics require an understanding of stars or stellar properties and so the study of nuclear reactions in the Universe is at the forefront of nuclear astrophysics.
Studying the reactions that occur in stars would seem to be a simple proposition because they occur at low energies and thus do not require large accelerators or intricate detector setups. However, such measurements are made exceedingly difficult by the fact that these energies are well below what is required to overcome the electrostatic repulsion between the positively charged nuclei. Thus, stellar reactions proceed via quantum-mechanical tunnelling, which leads to reaction timescales on the order of millions to billions of years during the quiescent phases of stellar evolution – and sets the scale for stellar lifetimes. In addition, the most interesting reactions to measure are often the slowest because they set timescales or limit energy production. As a result, direct measurements of astrophysically-interesting reactions require specialised accelerators, detectors, and techniques for reducing backgrounds. Even so, most reactions can only be measured at energies above the astrophysical region, and then the experimental cross-sections must be extrapolated to stellar energies using well-understood reaction theories, constrained by as much experimental information as possible.
Stars begin their lives by converting hydrogen into helium through reaction chains and cycles. Later, helium is fused into carbon and oxygen. Subsequent nuclear burning stages will produce nuclei up to the iron-nickel region. Helium burning in evolved stars also releases neutrons that, in turn, initiate nuclear reactions and thereby create about half of the elements heavier than iron. The remaining heavy elements are thought to be produced in the extreme environments of supernova explosions or neutron-star mergers. Nuclear reactions also power transient phenomena such as classical novae and X-ray bursts. A varied tapestry of nuclear processes is woven through these sites and scenarios, but the focus here is on the tools needed to study quiescent hydrogen and helium burning.
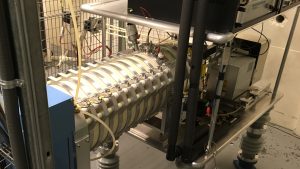
Experimental challenges
As mentioned above, the energy scales are low, on the order of a few kiloelectron volts (keV) to a few hundred keV, but the correspondingly low count rates necessitate very intense beams of protons and alpha particles. Reaction products (usually gamma rays, alpha particles, or neutrons) need to be detected with high efficiency, but detectors must also be compact in order to be shielded against the background.
Background sources include naturally occurring radioactivity from the U and Th decay chains and 40K, cosmic rays, and beam-induced events arising from trace contaminants in targets or vacuum systems. Cosmic-ray backgrounds can be significantly reduced by placing experiments underground, as has been demonstrated by for example, the LUNA1 and CASPAR2 collaborations (other underground facilities are planned or under construction). Note, however, that the ability to distinguish a signal from background depends linearly on the signal rate and on the statistical fluctuations of the background, which vary as the square root of the background rate. Thus, the approach that we have taken in constructing the Laboratory for Experimental Nuclear Astrophysics (LENA) has been to maximise the signal rate by producing extremely high beam currents.
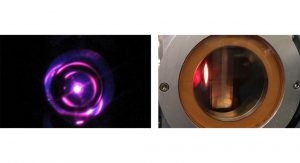
The Laboratory for Experimental Nuclear Astrophysics (LENA)
LENA is one of three accelerator facilities at the Triangle Universities Nuclear Laboratory (TUNL, a consortium of Duke University, North Carolina Central University, North Carolina State University, and the University of North Carolina at Chapel Hill). As originally constructed, LENA consisted of two accelerators, a high-current 230-kV accelerator3 based on an electron-cyclotron resonance (ECR) ion source and a 1MV, model JN van de Graaff accelerator. The ECR accelerator produced proton beam currents in excess of 2mA on target, making it the most intense low-energy accelerator for nuclear astrophysics worldwide (see Figs. 1 and 2).
Another important component of LENA has been a gamma-ray spectrometer consisting of a large-volume high-purity Ge detector surrounded by an annulus of 16 NaI(Tl) crystals, permitting coincidence, energy, and multiplicity requirements to be set. When combined with the beam current from the ECR accelerator, the sensitivity of LENA rivalled that of underground facilities for reactions where a coincidence condition could be imposed.4 The JN accelerator was used to extend measurements to higher energies and to probe systematic effects such as target composition and purity.
Note the past tense used in the previous paragraph. That is because LENA will be replaced by an entirely new facility. The ECR accelerator is being upgraded to produce target currents for protons of up to 20mA (corresponding to 4.6kW of beam power at full energy). Although this current is far in excess of what a typical target can withstand, the goal here is to operate the ECR accelerator in pulsed mode with 1-2mA equivalent continuous beam. The advantage of this type of operation is that environmental and cosmic-ray backgrounds will be reduced by a factor equal to the reciprocal of the duty cycle. For example, a 10% duty cycle would reduce these backgrounds by a factor of ten without any additional shielding. When combined with active and passive shielding and high beam currents, the net result is an experimental sensitivity to gamma rays below 3 MeV that is comparable to that achieved in a deep, underground location, provided that the reaction of interest emits two or more gammas (thus permitting a coincidence requirement).
The ECR accelerator will be combined with a 2-MV Singletron accelerator (designed and built by High Voltage Engineering Europa B.V.). This is an entirely new design, combining an ECR ion source with a fast beam-buncher/chopper system, producing high beam intensities (DC currents of 0.4-0.5 mA for E <1MeV and 2mA for E>1MeV) with pulsing frequencies up to 4MHz and pulse widths of 2-20ns. Pulsing again can be used for background reduction or for neutron spectroscopy via time-of-flight. The layout of the new laboratory is shown in Fig. 3. The beam transport for each accelerator has been designed to maximise the transmission of the beam to the target while minimising losses in intensity. This is a critical consideration given the high beam currents expected from both accelerators. Construction of the new facility is scheduled to be completed in the summer of 2021.
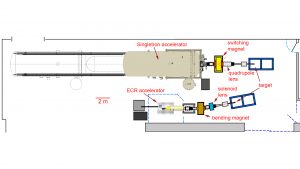
Experimental programme
The globular clusters in the halo of our galaxy are the oldest galactic objects for which reliable ages have been determined and they are thought to be the first structures to form in the early history of the galaxy. Thus, they provide information about the early galaxy and their locations, motions, and ages are important ingredients in understanding the evolution of the galaxy. At the same time, they also present fascinating puzzles. For example, anticorrelations involving C vs. N, O vs. Na, Mg vs. Al, and Mg. vs. K are not easily explained and hint at a more complex history than previously believed. In this regard, the cluster NGC 2419 (see Fig. 4) is particularly interesting because its abundance signatures appear to arise from an unknown process.5
Initial measurements at the new LENA facility will focus on the key reactions that are thought to give rise to these anomalous abundances. However, before any measurements are contemplated, it is important to understand how individual reactions can affect the observed abundances. For this, we make use of reaction-network calculations using the STARLIB reaction-rate library. STARLIB is available at https://starlib.github.io/Rate-Library/ and is the only rate library that incorporates statistically meaningful uncertainties. This, and other tools developed by our group allow us to quantify the impact of laboratory measurements on stellar models.
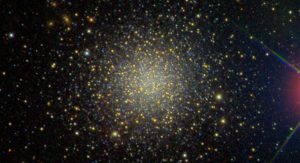
Summary
Experimental nuclear astrophysics is a rich field and the extremes in stellar environments have challenged the community to advance the state of the art in accelerators and detectors. In this article, I have tried to describe one aspect of the endeavour, namely direct measurements of reactions that are important for quiescent stellar burning. I have also described one approach to these measurements, the new LENA facility that will begin operations in 2021.
Acknowledgements
The author thanks his collaborators C. Iliadis, R.V.F. Janssens, and R.L. Longland. This work was supported in part by the Office of Nuclear Physics at the U.S. Department of Energy.
References
- Prati for the LENA collaboration, ‘The LUNA-MV Facility at Gran Sasso’. J. Phys.: Conf. Ser. 1342, (2020), 012088
- Robertson, et al., ‘Underground Nuclear Astrophysics Studies with CASPAR’. Eur. Phys. J. Web of Conferences, 109, (2016), 09002
- See A. L. Cooper, et al., ‘Development of a Variable-Energy, High-Intensity, Pulsed-Mode Ion Source for Low-Energy Nuclear Astrophysics Studies at LENA’.Rev. Sci Instr. 89, (2018), 083301-1 through 083391-10
- Q. Buckner, et al., ‘High-intensity Beam Study of 17O(p,γ)18F and Thermonuclear Reaction Rates for 17O+p’, Phys. Rev. C 91, 2015, 015812-1 through 015812-15
- Iliadis et al., ‘On Potassium and Other Abundance Anomalies of Red Giants in NGC 2419’. Ap. J. 818 (2016), 98
Professor Arthur E Champagne
University of North Carolina at Chapel Hill
and TUNL
+1 919 660 2607
artc@physics.unc.edu
aec@tunl.duke.edu
https://tunl.duke.edu
Please note, this article will also appear in the fourth edition of our new quarterly publication.