Professor David H Reitze, Executive Director of the Laser Interferometer Gravitational-wave Observatory (LIGO) Laboratory at the California Institute of Technology, discusses the importance of gravitational wave research and the huge scope for further discovery as a result of innovation.
The National Science Foundation Laser Interferometer Gravitational Wave Observatory (LIGO), based in the US, is the world’s largest gravitational wave observatory and one of the world’s most sophisticated physics experiments. Designed to explore the field of gravitational wave astrophysics through the direct detection of gravitational waves predicted by Einstein’s general theory of relativity, LIGO consists of two laser interferometers located thousands of kilometres apart in Livingston, Louisiana, and in Hanford, Washington.
LIGO’s multi-kilometre-scale gravitational wave detectors use laser interferometry to measure the minute ripples in space-time caused by passing gravitational waves from cataclysmic cosmic events such as colliding neutron stars or black holes. LIGO made its first detection of gravitational waves, generated by a pair of colliding black holes some 1.3 billion lightyears away, in 2015. Since then, LIGO’s scientists and engineers have continued to improve the detectors’ sensitivities and have gone on to detect many more gravitational waves. Improvements are being continually made to refine and upgrade the LIGO detectors to enhance their sensitivity and, in turn, greatly increase the scope for discovery. LIGO is funded by the National Science Foundation and operated by the California Institute of Technology (Caltech) and Massachusetts Institute of Technology (MIT).
The Innovation Platform spoke to Professor David Reitze from Caltech’s LIGO Laboratory to find out about the existence of gravitational waves, LIGO’s work, and the future of gravitational wave research.
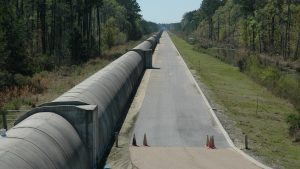
Can you provide a brief overview of what gravitational waves are, what we have learnt about the cosmos from observations of gravitational waves so far, and what LIGO’s research aims to achieve?
As the name implies, gravitational waves are related to the way that gravity propagates through space. They were first predicted by Albert Einstein in his general theory of relativity over 100 years ago. Einstein’s insights allowed us to understand that space and time are, essentially, manifestations of the same thing, as are matter and energy. We have since discovered that matter and energy are interchangeable and, when matter and energy are put into space, they can accelerate and generate a ripple in space-time – known as a gravitational wave. To use an example to explain, when you have two gravitationally bound objects, like the Earth going around the Sun, both objects are accelerating and, as they do so, release energy in the form of gravitational waves.
LIGO looks for gravitational waves from compact astrophysical objects such as colliding black holes. These are very massive, and very compact objects. A black hole that is, for example, 30 times the mass of the Sun has a diameter of nearly 200km. This is a huge amount of mass collapsed into a very tiny sphere. When you have two of those things orbiting around one another, the amplitude of the gravitational wave produced (dependent on where you are looking at it, in this case about 500 million lightyears away) is 10-21. Therefore, we are looking for an amplitude that is one part in one billionth of one trillion.
Physically, gravitational waves are actually ‘strains’. To illustrate, if you have a bar of aluminium that is one metre in length and you then compress that bar, the atoms squeeze but with some resistance. As I apply a force, the bar will get shorter by a tiny amount. The amount by which it gets shorter relative to the original length is the strain. Space behaves in exactly the same way. Massive objects that are moving and accelerating strain the space-time, so the space-time slightly stretches or squeezes. This is important for understanding how you detect gravitational waves. If I shoot a gravitational wave at something, the horizontal axis to either side of the direction the gravitational wave is travelling would stretch by a tiny amount, whereas the vertical axis would compress by equally tiny amounts at one instant in time. The stretching and squeezing depend on time; at other times the vertical axis is stretched while the horizontal axis is compressed. This change, the ‘strain’ in space-time, is what you must measure.
The reason why gravitational waves are so important and why we want to detect them is because they are a completely new way of looking at the Universe. Most of what we know about the Universe comes from telescopes looking at stars. Telescopes look at light, whereas gravitational waves are produced by accelerating matter, so they are complementary to what we can see with telescopes.
So far, we have learnt many things. The first direct detection of gravitational waves happened in 2015 and we discovered that black holes could exist in pairs. We also learnt that a whole new ‘heavier’ class of stellar mass black holes exist.
We can also directly measure the waveform of these gravitational waves. From this, we learn a lot about the nature of the black holes, including their mass and how much they are spinning. They tell us that the black holes that we see in the Universe are indeed the black holes that were predicted by Albert Einstein over 100 years ago.
We have also made some fantastic discoveries with neutron stars. Black holes and neutron stars both originate from the end stages of stellar evolution. A massive star in the Universe will burn through its fuel, hydrogen, and higher, heavier elements before collapsing. Depending on how massive it is, it will either collapse into a white dwarf, a neutron star, or a black hole. As the name implies, a neutron star is primarily made up of neutrons and is a very compact object. These neutron stars tend to be about 1.2 to 2 times the mass of the Sun, but they are packed into a sphere that is about 25km in diameter. These very dense objects are extremely interesting and have been studied for a long time using mostly radio telescopes, because they emit radio pulses. We see these systems in binary systems and, in particular, we can watch them as they collide.
In 2017, we observed our first binary neutron star collision which enabled us to infer important information, including its diameter and how massive it is. In addition, when these neutron stars collide, they produce the most spectacular firework show in the Universe. As they are moving very fast and have lots of matter, they create cataclysmic explosions that produce gamma ray bursts, optical light, X-rays, and radio waves. Essentially, they radiate across the electromagnetic spectrum. Using conventional astronomical telescopes that look for light, astronomers were able to find this object very soon after we detected it, and measured all kinds of different interesting properties, including that many of the heaviest elements in the periodic table come from the collisions of these neutron stars.
There are lots of really interesting and new things that LIGO has achieved so far. Our ultimate goal is to bring a new kind of astronomy to the world, to be able to study very cataclysmic objects in a unique regime.
How is innovation helping to further LIGO’s research?
Einstein had a very complicated relationship with gravitational waves and, once he believed they existed, he did not think that they would be physically interesting, because the effect is so tiny. This is where innovation comes in. Einstein did not know about lasers, control systems, or the kind of vacuums we are able to build today – all the things you must consider to be able to build a gravitational wave detector.
LIGO is an interferometer, which is a physical device that measures differences in the lengths of two different arms that are perpendicular to one another, by measuring the difference in the time of flight of light. We use an ultrastable laser to generate the light, and a beam splitter to split the light, which causes the light to go in two different perpendicular directions. We then have mirrors that reflect the light back and, depending on the presence of the gravitational wave, the round-trip time (the amount of time it takes for the light to travel from the beam splitter to one mirror and back and then the beam splitter to the other mirror and back) is different. LIGO measures that difference.
All the technology that you need to construct a gravitational-wave interferometer had to be developed. At the base level, the innovation was new and more stable lasers, more precisely polished mirrors, and huge vacuum systems. The interferometer itself cannot be built in a laboratory as its arms are 4km long. Innovation has been vital in enabling us to build these detectors that can confidently detect gravitational waves.
We are constantly innovating. One of the newest innovations we have borrows ideas from quantum physics, to improve our detector sensitivity. LIGO and gravitational wave detectors are some of the best examples of how you can use quantum mechanical manipulation of light to do something useful – in our case, to improve our ability to detect gravitational waves.
Another aspect of our innovation is mirrors. We have the world’s most precise mirrors and, in order to make them, the people we work with have had to develop the best optical metrology. Very recently, we have been developing new kinds of optical coatings on our mirrors to improve their performance by minimising intrinsic vibrations.
We are also innovating in computing by using Machine Learning (ML) to implement some of our search algorithms.
The bottom line is that, in our field, we have to constantly innovate to improve our detectors.
How important is international collaboration in furthering gravitational wave science?
It is critical. The concept of LIGO was started around 45 years ago by a group of scientists at MIT (primarily Rainer Weiss) and Caltech (Kip Thorne and Ron Drever), who initially worked independently before merging forces. It became very clear over time that, because of the scale and complexity of these detectors, a huge ecosystem of scientists and engineers was required to design, build, and operate the detectors, and develop the computer algorithms needed to search through the data produced. Some of the key components of the LIGO detectors were built by our international collaborators in the UK, Germany, and Australia. Expertise from the global community is essential to be able to do what we do.
At the same time that LIGO was being born in the US, many similar efforts were also underway in Europe. As time went on, it was realised that collaboration was crucial in order to carry out the amount of work that needed to be done. In 1997, we formed the LIGO Scientific Collaboration which, at the time, comprised 250 people from the US, Europe, Australia, Russia, and more. This collaboration is now made up of over 1,500 people, with members from around 25 different countries, working on a broad spectrum of science.
In addition to LIGO, there are other gravitational wave projects that have built ground-based detectors. In Italy, there is a detector called Virgo that was developed and built independent of LIGO. In 2007, however, we joined together and began collaborating after recognising that you can gather a lot more information by operating as a network. We have also joined forces with the Kamioka Gravitational Wave Detector (KAGRA) in Japan.
Collaboration is so important because telescopes are very good at pinpointing the location of an object, whilst gravitational wave detectors are not. If a gravitational wave source is at one point in the sky, it will register a signal if it is powerful enough. If it is at a different point in the sky, it will also register a somewhat weaker signal, so you do not know where it came from. However, by using multiple detectors, in particular three, you can triangulate. By measuring the difference in arrival time with the gravitational wave, you can localise the source. This will not be very precise, and certainly not as precise as telescope detections, but you will at least be able to inform your colleagues that run telescopes of where to look.
What other research is being done in the field? How do LIGO’s observations complement this?
There are more detectors that we believe are going to be coming online. We are working with laboratories in India to build a replica of the US LIGO detectors to enable us to obtain more information about the nature of the source by having more detectors on it.
In terms of other research, we already talked about multi-messenger astronomy – the idea that you can look at gravitational wave objects with telescopes and they also look like gamma ray emitters, radio emitters, and X-ray emitters. There is a huge amount of work going on in this area now. A lot of astronomers are very interested in trying to follow up LIGO detectors. There are a lot of proposals for satellite missions to look for ultraviolet emissions from objects in space to try to follow up these gravitational wave events. The field is fairly young – we have only been directly detecting gravitational waves for seven years now.
We are also working with neutrino detectors. It is quite possible that there are sources that produce neutrinos and gravitational waves, and that we may be able to see those things in coincidence.
There are a number of big outstanding questions in physics and cosmology. What is the nature of dark matter? What is dark energy? Why is the Universe accelerating and entering another inflationary epic? Gravitational waves could shed some light on the nature of dark matter. For example, it could be a type of tiny primordial black hole.
There is also a lot of work going on to leverage new ways of computing. For example, Artificial Intelligence (AI) and Machine Learning are things that we have heard a lot about, because they are very useful in image recognition, but they are also very useful in controlling complex interferometers. We are looking at applying ML methods to improve the way we control our interferometer and to achieve better recognition of gravitational wave sources. Finding a gravitational wave, because of the nature of the amplitude and because the interferometers themselves produce a lot of noise, is very difficult. Therefore, trying to write algorithms that can sift through very noisy data and identify a gravitational wave is something we are always pushing the boundaries on.
What does the future look like for gravitational wave research? What is the ultimate hope for such observations?
I think the future is very bright. The field has really exploded in the last seven years. It was once a question of whether it was even possible to detect gravitational waves. Now, when our detectors are running, we detect them once a week. We are continually trying to improve on this, and we are set to enter a run next year where we expect to detect a gravitational wave once every two to three days. It has not become completely routine, but it is now a very established field looking at this new sector of the Universe.
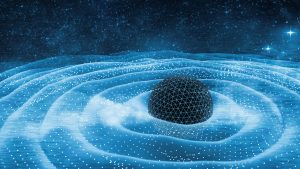
In the short term, we always want to improve our detector sensitivity. Right now, we can see gravitational wave sources for black holes up to a few billions of lightyears away, which is not actually that far in the Universe. For neutron stars, we cannot see very far at all. By improving the sensitivity, we will be able to go further into the Universe, opening up a lot more possibilities for detection.
Ultimately, we are limited by the length of the arms of our interferometers. The arms of the LIGO interferometers are 4km, whilst VIRGO has slightly shorter arms at 3km. There is no fundamental reason why you cannot build an interferometer that is ten times longer and has 40km arms. It is complicated and there are lots of technical challenges that you need to understand and overcome, but it is achievable now that we know how to detect gravitational waves. There are plans to develop these new generations of gravitational wave observatories in the next decade.
In Europe, there is the Einstein Telescope, which is a very novel design and is a much bigger interferometer than the Virgo interferometer. In the US, we are working on the Cosmic Explorer, which is ten times the size of LIGO with 40km arms. By just making that simple change, you improve the reach of your detector by about a factor of ten. To me, this is quite remarkable. These detectors will be able to see the edge of the Universe. They will be able to see every black hole merger that ever happened and even the epic before stars were formed. Within 15-20 years, we will go from just seeing a gravitational wave detector to seeing the entire Universe in gravitational waves.
The other dimension is frequency. As with light, gravitational waves come in different wavelengths and, in LIGO’s case, short wavelengths mean thousands of kilometres. LIGO detects gravitational waves in the short wavelength band from compact objects. However, there are longer wavelength gravitational waves that are produced by more massive objects, such as very massive black holes, that are up to hundreds of thousands of solar masters. LIGO or Virgo cannot see these because the frequency of their emissions is too low for us. Fortunately, there are detectors that are planned to do that. The best example is the Laser Interferometer Space Antenna (LISA), led by the European Space Agency (ESA) with participation by NASA, which is set to be launched into space within the next decade. The idea is to make the same kind of detector that we have on Earth, but in space. Instead of having 4km arms, their arms are going to be 2.5 million kilometres. This is impressive technology.
Is there anything else you would like to mention?
I want to emphasise that LIGO was a big risk when it was conceived. It is a highly ambitious feat to build a detector with such large arms, one of the world’s largest vacuum systems, the world’s most stable laser, and the world’s best mirrors that will be suspended by glass threads and will be extremely isolated from the ground. In fact, there was a general sense that gravitational waves would never be detected, not only because it was too hard to detect them, but because people did not know several decades ago what the most promising sources were. This is where governments make a difference. LIGO would have never been funded without the support of the US Government, which approached the National Science Foundation (NSF) for funding. This was a high risk bet that did carry the potential to fail, however, it also had the potential to be Nobel-Prize-winning science that could open a new window into the Universe. This took a lot of courage from the NSF, but it has paid off.
Please note, this article will also appear in the twelfth edition of our quarterly publication.